Image above provided courtesy of: joiseyshowaa. "World Class Traffic Jam: Jersey Turnpike Version". March 6, 2016. Online image. Flickr. March 1st, 2024. https://www.flickr.com/photos/joiseyshowaa/25422136442
TL;DR: Air pollution is a pressing global issue with wide-ranging implications for human health, environmental well-being, and climate change. Among the myriad air pollutants, black carbon stands out as a significant contributor to the complex web of air quality and climate concerns we face. In this post, we asked Drew Hill, Data Science & Applied Research Lead at Aethlabs to help us take a deep dive into black carbon air pollution, exploring the science behind this air pollutant, its origins, its impacts, and the urgency & importance of accurate black carbon measurement.

If you want to learn more about black carbon and the applications for black carbon measurements, register for our upcoming webinar Beyond Mass Concentration: The Synergy of Black Carbon and Particulate Matter Measurements in Air Quality Monitoring.
What is black carbon air pollution?
Black carbon, commonly referred to as soot, is the dark light-absorbing and heat-absorbing carbonaceous particulate pollutant released during incomplete combustion. The primary sources of black carbon vary by location but typically include diesel engines, coal-burning, wood stoves, agricultural burning, and wildfires.

Black carbon is a critical component of particulate matter air pollution around the world — often accounting for between 5-25% of measured PM2.5 concentrations according to the Spartan Network. Recent evidence has shown a greater impact from black carbon on health than PM2.5 alone, and, despite a relatively short atmospheric lifetime of about 4-12 days, black carbon is estimated to be the second most important human-emitted climate pollutant – only CO2 is more salient. For such a small, short-lived pollutant, black carbon exhibits profound impacts on our health and climate.
Black carbon air pollution sources and formation
Common sources of black carbon air pollution include diesel vehicles, household cooking and heating, industrial processes, and wildfires. Because black carbon is produced during incomplete combustion, it is typically emitted alongside other particulate matter and key pollutants like carbon monoxide, CO2, and volatile organic compounds. Policies that successfully target black carbon emissions reductions almost always result in benefits from reducing these co-pollutants, as well.
Black carbon sources vary considerably by location. In Asia and Africa, emissions profiling work suggests that the residential burning of solid fuels like wood account for 60-80% of anthropogenic (i.e., human-produced) black carbon emissions. In Europe and North America, diesel engines contribute approximately 70% of anthropogenic black carbon emissions. Vulnerable communities, particularly those using biofuels or coal for cooking, bear a disproportionate burden of the health consequences associated with black carbon exposure — approximately 3.2 million annual deaths are caused by the use of indoor cookstoves worldwide, according to the World Health Organization, including nearly a quarter of a million deaths in children every year.
As wildfires grow in intensity and frequency due to climate change, they account for an increasing portion of total black carbon emissions worldwide. Wildfires release large amounts of particulate matter rich in black carbon into the air and, unfortunately, into the lungs of people living both nearby and very far away. Scientists estimate that, since 2010, the increased frequency of wildfires on the US West Coast has reversed the substantial gains in air quality made in recent decades of stringent policy implementation, increasing western US black carbon concentrations by 86% and increasing the amount of black carbon in PM2.5 by ~ 2.4% annually across the country.
Where is black carbon air pollution most prevalent?
The prevalence of black carbon air pollution varies around the world, with distinct patterns influenced by regional economic development, regulatory measures, and emissions sources.
More than 75% of global black carbon emissions originate from Asia, Africa, and Latin America, primarily due to open biomass burning and residential solid fuel combustion in developing regions that lack comprehensive air quality regulations. The fact that such a large proportion of global anthropogenic emissions comes from developing economic regions is due, in part, to the co-reductions in black carbon emissions that have been realized in many developed countries over recent years under increasingly strict air quality regulations. Still, given the substantial effects of even relatively small black carbon emissions, developed and developing nations have a long way to go in reducing black carbon health & climate emissions.

Asia, in particular, emerges as a hotspot for black carbon pollution, with China and India topping the list for the highest national BC emissions. In the summer, these two countries collectively contribute 76% of total black carbon emissions in Asia. A study examining air pollution in over 600 Chinese cities revealed a concerning annual mean black carbon (BC) concentrations, reaching up to 10 μg/m3 in urban areas and up to 4 μg/m3 in rural sites — values with concerning implications for public health in the region.
In contrast, developed countries, such as the United States, have made significant strides in reducing anthropogenic black carbon emissions, which predominantly arise from diesel vehicle emissions. California, for instance, has witnessed a remarkable 90% decrease in BC emissions over the past 50 years, attributed to stringent emission standards and restrictions on diesel engines and biomass burning, and despite an increase in overall state-wide diesel consumption. The United States as a whole, which, including wildfires, is responsible for approximately 8% of global black carbon emissions as of ~ 2016, was projected to reduce anthropogenic emissions by 42% from 2001 to 2020 primarily due to new diesel-vehicle regulations. Regulation works!
In Europe, while specific monitoring of the black carbon aspect of particulate matter is not (yet!) mandatory, many air quality monitoring networks measure black carbon due to its historical association with 'black soot.' Forward-thinking countries like France, with, as of 2021, a national monitoring network of at least 19 black carbon monitors at 11 sites, are leading the way.
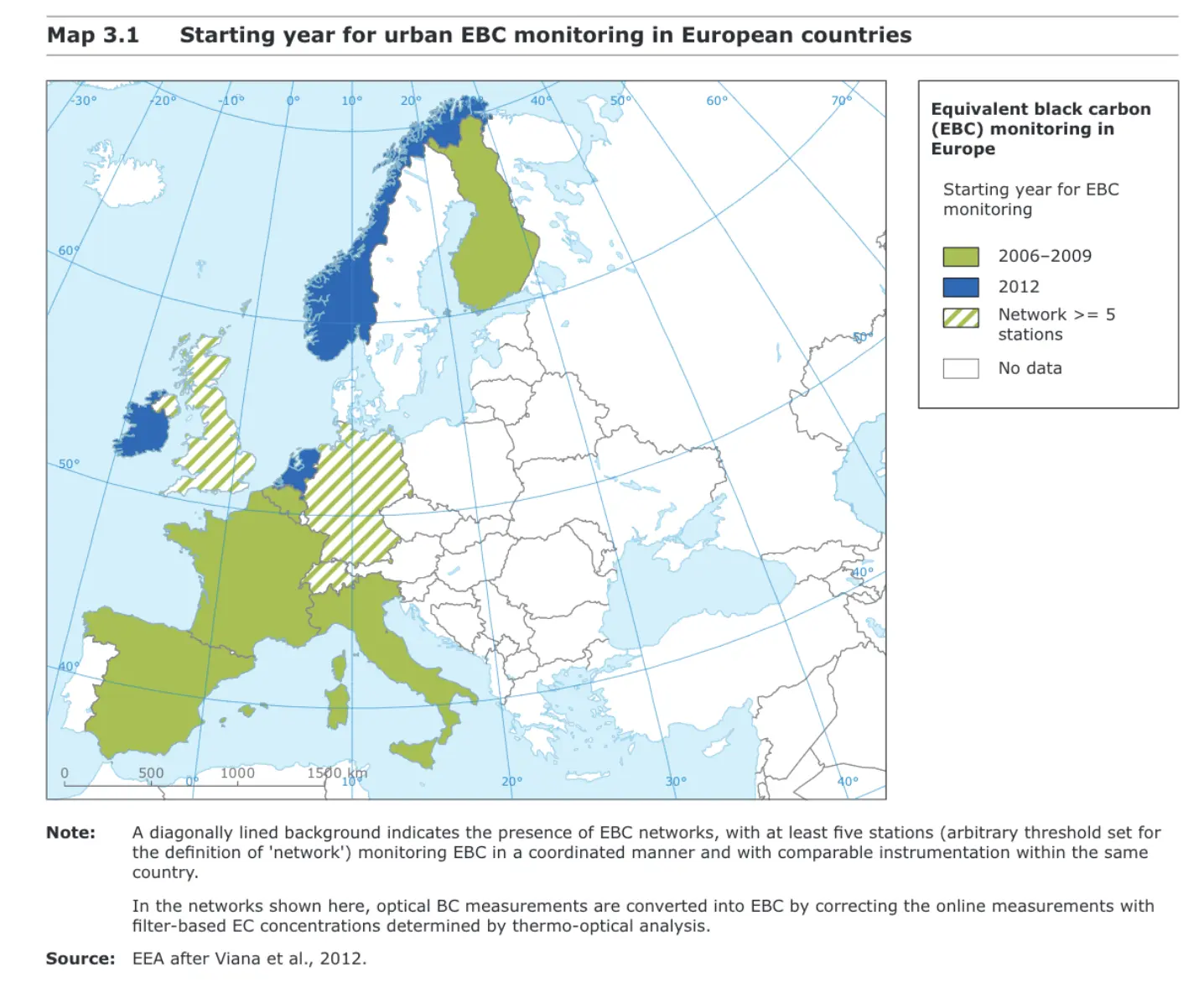
One significant source of black carbon emissions cannot easily be linked to a specific country or region, as it is, by its very nature, international: shipping. If it were a country, the maritime shipping industry would rank as the sixth-largest contributor to greenhouse gas (GHG) equivalent emissions globally, surpassing Germany, with 7-21% of the industry’s emissions coming from black carbon alone. The industry’s impact is made greater by the direct effect these emissions can have on global albedo (solar light/heat reflectivity) when emitted during trans-arctic voyages and deposited onto the surfaces of pristine ice cap snow. Despite international efforts, the UN's International Maritime Organization (IMO) has yet to regulate black carbon emissions from maritime sources, posing an additional challenge to global black carbon reduction goals.
These global patterns highlight the complex interplay of economic development, regulatory frameworks, and regional sources in the prevalence of black carbon air pollution, and set the stage nicely for a discussion of the need for increased policy-oriented measurement.
Why is it important to measure black carbon?
Black carbon air pollution harms human health, exacerbates climate change, and raises questions of environmental justice.
Human health impacts of black carbon
To call black carbon particles tiny does their microscopic size a disservice: at < 1 micrometer in diameter, a black carbon particle freshly emitted from diesel combustion typically measures in at about 100 times smaller than the width of a human hair. Given this small size, black carbon particles, when inhaled, can sneak past our body's defenses and find their way into the deepest parts of our lungs, even translocating directly into our bloodstream and critical organ systems. The result is a spectrum of health issues, including lung and heart diseases that can lead to premature death.
Many of the specific mechanisms by which black carbon affects our health are still being uncovered. Still, a robust body of research associates black carbon exposure with numerous health concerns and identifies a series of immunological and toxicological pathways of action. From childhood asthma and lower respiratory issues to low birth weights and increased risks of heart attacks and lung cancer, the implications are undeniable.
One way to think of the hazard posed by exposures to black carbon is in relation to those of PM2.5, which are well-characterized and highly publicized. Ambient PM2.5 is responsible for approximately 6.4 million deaths every year, primarily due to ischemic heart disease, stroke, and COPD, among other illnesses (check it out for yourself using this powerful web tool for visualizing the global burden of disease data from the Institute for Health Metrics & Evaluation at the University of Washington).
Amplifying the urgency of such health effects, the per-capita rate of PM2.5-related mortality is heavily skewed toward low-income countries, with a rate nearly 4 times higher than in high-income countries. Recall from earlier in this post that, globally, black carbon comprises somewhere between 5-25% of PM2.5. Using a direct 1:1 hazard relationship for black carbon: PM2.5, we can (very broadly and unscientifically) estimate somewhere between 320,000 - 1,600,000 annual deaths from black carbon exposure every year.
But the relationship between black carbon exposure and health effects is often demonstrably stronger than for PM2.5 alone! A 2021 study, for example, identified 50% greater odds of asthma prevalence associated with per-mass concentrations of black carbon than for concentrations of PM2.5. Another study – this time of asthmatic school children in the Bronx (New York City, USA) – attributed an increase in black carbon exposures with upwards of 100% greater relative risks of adverse asthma outcomes while effects from personal PM2.5 exposures were completely insignificant.
Notably, such alarming health concerns have been identified even in seemingly low-pollution environments. A recent study conducted in Swedish cities found the risk of stroke to increase by 4 percent for every 0.3 μg/m3 of black carbon measured, highlighting the pervasive and far-reaching health effects of black carbon on human health.
Environmental and climate impacts of black carbon
Black carbon’s impacts extend beyond direct human health concerns, representing significant risks to the natural environment. One of its far-reaching consequences is its effect on plant life. By reducing sunlight, black carbon can impact the vitality of plant ecosystems, impacting their health and productivity. This diminution in sunlight also contributes to haze pollution episodes, creating a veil that alters the visual landscape and affects the overall well-being of plant life.
Beyond the immediate environment, black carbon plays a noteworthy role in contributing to climate change. Black carbon’s dark color and unique ability to absorb solar heat allow it to warm the air, making it a notable climate-forcing agent. It's worth highlighting the staggering statistic that underscores the climate change potency of this pollutant: black carbon has a warming effect 460-1,500 times stronger than CO2 per unit mass. In fact, black carbon is second only to CO2 in its overall impact on climate change (which, in addition to climate forcing potency, accounts for things like the actual amount emitted around the world and atmospheric lifetime [< 2 weeks for black carbon and 300 - 1,000 years for CO2]). Certainly addressing black carbon emissions is an integral component of broader climate change mitigation strategies.
The localized warming of air imposed by the presence of black carbon can lead to rapid changes in rain and cloud patterns in the atmosphere, influencing regional circulation and rainfall patterns. Such alterations have tangible repercussions for agriculture, particularly severe in Asia and Africa, where monsoons play a pivotal role in sustaining crop growth. Black carbon’s localized warming effects also have direct consequences for glacial melt – and related drinking water supply – in South Asia. Black carbon emitted in South Asia will, due to regional weather patterns, be transported to the Tibetan plateau where atmospheric processes, transport timing, and black carbon’s short atmospheric lifetime conspire to settle it onto the plateau’s glaciers. Once settled, black carbon darkens the glaciers’ pristine white surfaces, reducing their ability to reflect the sun’s warming rays and actively absorbing solar heat, ultimately shrinking the glaciers upon which billions of people rely.
Similar consequences extend even to polar regions, where the deposition of black carbon in the Arctic disrupts the delicate balance of snow and ice and, in turn, reduces the Earth’s ability to continue reflecting solar radiation back into space. To safeguard the Arctic environment, initiatives like the EU-funded Action on Black Carbon in the Arctic have emerged, aiming to collectively reduce black carbon emissions and foster international cooperation.
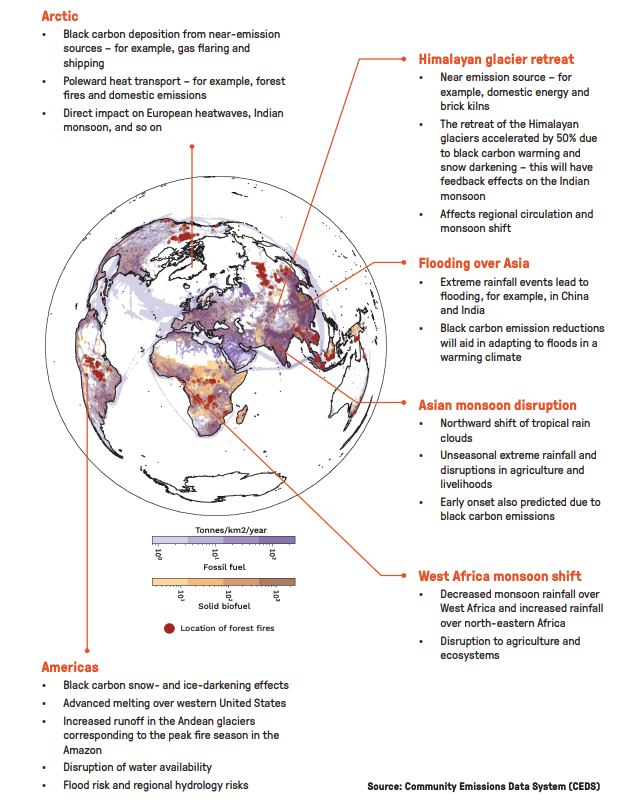
Black carbon is a prime example of how taking action to reduce air pollution can yield climate co-benefits – the incomplete combustion processes that produce black carbon also invariably produce a large number of other pollutants. Recognizing the urgency of addressing black carbon emissions, the Clean Air Fund emphasizes the critical importance of this effort for both fast climate mitigation and building resilience against the impacts of climate change in their report The Case For Action on Black Carbon.
Environmental justice implications of black carbon air pollution
The most severe health consequences of black carbon often disproportionately affect vulnerable and marginalized communities – particularly indigenous groups – and, in many parts of the world, women and children. For example, black carbon is a critical element of the air pollution emissions resulting – often directly in peoples’ kitchens – from the residential burning of solid fuels and kerosene that occurs in the homes of billions of people and causes millions of deaths every year.
Industrial sources of black carbon, such as those originating from coal-burning plants and brick kilns, can emerge as the primary contributors to air pollution in certain urban areas, adversely affecting the well-being of residents of lower socioeconomic status who often live close to these industrial operations. Black carbon from diesel engine emissions – which accompany many industrial processes and, of course, major roadways – also tend to be more highly concentrated in these disadvantaged areas inhabited by marginalized communities.
Source apportionment insights
Black carbon’s physical characteristics facilitate a uniquely high climate impact, induce potent health effects, and ultimately make its atmospheric presence powerful but short-lived (i.e., an ideal target for mitigation). As will be discussed later in this post, the properties that underpin these traits also allow us to measure black carbon in real-time with high reliability and source-specific attribution, providing continuous insights on source apportionment and increasing the efficiency and effectiveness of data-driven policy actions.
How is black carbon air pollution regulated?
The regulatory landscape for black carbon air pollution is a fairly loose patchwork compared to the regulations in place for many other pollutants, such as those managed as criteria pollutants in the United States.
In the United States, while black carbon is indirectly regulated as part of particulate matter (PM) under the National Ambient Air Quality Standards (NAAQS) standards for PM, as mandated by the Clean Air Act, it is not itself a criteria pollutant. Currently, there exist no direct regulations on ambient black carbon concentrations in the US.
The US Mine Safety and Health Administration (MSHA) limits certain occupational exposures to diesel particulate matter – an aerosol mixture often rich in black carbon – based on the measurement of elemental carbon (a term often used interchangeably with black carbon or with a near-1 translation factor). Bolstering US efforts to reduce exposures to black carbon-related diesel emissions is the Diesel Emission Reduction Act (DERA), a program initiated in 2005 under the United States Environmental Protection Agency (USEPA). DERA operates as a grant program with a mission to reduce diesel emissions, offering support for initiatives like replacing old diesel engines in school buses, thereby indirectly addressing black carbon emissions.
Across the Atlantic, the European Union (EU) has implemented measures to combat black carbon within the framework of the National Emissions Ceiling Directive, established in 2016. This directive compels Member States to prioritize reductions in black carbon when implementing measures to address PM2.5. The key sectors targeted for PM regulation, including road and non-road transport, solid fuel domestic combustion, and open burning of agricultural waste, align with those identified as principal emitters of black carbon, ensuring robust measures for its abatement. However, it's noteworthy that the EU currently lacks a specific metric for black carbon, incorporating it instead as part of its broader regulation of particulate matter.
In both the United States and the European Union, the regulatory frameworks emphasize a comprehensive approach, addressing black carbon both directly and indirectly within broader initiatives aimed at mitigating the impacts of air pollution. As we continue to refine and expand these regulatory strategies, it would be wise to incorporate measures that more directly address black carbon itself given its noteworthy impacts on human and environmental health.
How is black carbon air pollution measured and how can these measurements be used to identify pollution sources in real-time?
The first step to better understanding and managing black carbon air pollution is measuring it. Accurate black carbon measurement allows us to uncover its connections to human well-being, its role in altering climate patterns, and the disproportionate burden it places on marginalized communities. Measurement also allows us to track the progress of mitigation efforts, allowing us to identify implementation efficiencies or to change course when specific implementation paths prove ineffective.
Black carbon concentrations are typically communicated in micrograms or nanograms per cubic meter (µg/m3 and ng/m3, respectively). Black carbon measurement can be accomplished through a variety of methods and technologies that gauge its concentration in the air, though most commonly used approaches employ either thermal/optical methods or absorption photometry.
Thermal/optical methods produce estimates of elemental carbon (EC) and involve collecting particulate matter on a filter – often over extended periods of time– and subsequently determining the black carbon content (as EC) by heating that filter up to different temperatures and measuring what vaporizes along the way. This approach differentiates pure elemental carbon (more akin to, but not exactly, black carbon) from organic carbon (lighter-colored carbonaceous material). The result is a highly resolved quantification of the elemental and organic carbon composition of particulate matter on a sample, but is labor-intensive, expensive, and time-consuming, making real-time measurements difficult and impractical.
Absorption methods (or “optical” methods) are similar in principle to thermal/optical elemental carbon analysis (ECA): particles are collected on a filter and a laser (or similar light source) is used to assess the presence of black carbon. Black carbon is great at absorbing light at ~ 880nm (IR or near-IR light), but very few other particles commonly found in our atmosphere are. Thereby, if we measure the amount of 880 nm light that passes through a filter both before and after running an air sample through that filter, and if we find that the amount of light that can be transmitted through that filter decreases after the air sample has been run through it, then we a) know that something in the air sample that absorbs 880 nm light was deposited onto the filter and b) can assume that this 880 nm light-absorbing “something” is black carbon as the likelihood of any other 880nm-absorbing compounds existing in that air sample is negligibly low. The relationship is so reliable, that a main tenet of optical physics called Beer’s Law can be used to translate the amount of light “lost” into an estimate of the mass of black carbon particles deposited onto a filter during such an air sample.
While a number of creative and effective absorption-based measurement methods exist, the most widely used – likely due to its practicality, cost, ability to produce real-time measurements, and amenability to implementation in a portable instrument form factor – is called aethalometery. An aethalometer, like those comprising the AethLabs microAeth® product line, leverages Beer’s Law to optically measure black carbon concentrations as rapidly as every second to give users insights into immediate hazard information as well as long-term trends.
Some aethalometers can measure black carbon concentrations across multiple wavelengths, providing continuous insights on source apportionment and increasing the efficiency and effectiveness of data-driven policy actions. To understand how and why this works, you must first understand that black carbon tends to exist in complex carbonaceous aerosol mixtures. The composition of these mixtures depends on the fuels that were burned while producing them and, as such, information on composition can provide clues about what those fuels (i.e., sources) were.
The underlying science is a bit complicated, and we’ll discuss the methods in more detail later on in this post, but the gist is that fossil fuel combustion produces relatively “pure” graphitic black carbon, which absorbs light with equal relative intensity in near-infrared (IR) and near-ultraviolet (UV) wavelengths, while the carbonaceous aerosol mixtures produced by the burning of biomass like wood and agricultural residues tend to be rich in both black and organic carbon (OC), which absorbs near-UV light at a stronger rate than near-IR light. Optical black carbon monitors measuring at multiple wavelengths between the IR-UV range will capture this differential, providing information on the amount of BC vs. OC contained in a measurement.

An important phenomenon referred to as “filter loading effects” or “shadowing” can occur in optical absorption measurements under high levels of filter loading – when sampling filters are not frequently changed out or particularly high concentrations are sampled for extended periods. In essence, with enough sampling, particles will begin to accumulate on the filter in such a way that causes occlusion of the light absorption effects of other particles – think two overlapping shadows. Fortunately, a small number of approaches for mitigating such filter-loading effects have been developed, including the DualSpot® method deployed in several AethLabs products.
AethLabs partnered with Clarity to leverage the principles and benefits of multi-wavelength absorption methods and DualSpot® loading compensation to develop the Black Carbon Module, which is based on our microAeth® Black Carbon measurement technology and integrates seamlessly into the Clarity ecosystem. Designed to serve as an outdoor measurement point mounted on street poles and fence lines, this device is intended for prolonged multi-month measurement initiatives that demand minimal upkeep and rare on-site inspections.

The Black Carbon Module offers real-time measurements across 5 wavelengths (IR, Red, Green, Blue, and UV), using the optical properties of the carbonaceous aerosols emitted from fossil fuel (e.g. diesel) and biomass (e.g. wood smoke) combustion to quantify the source-specific concentrations of black carbon particles. The device’s DualSpot® loading compensation method leverages a sophisticated technique that corrects for optical loading effects and may also shed light on additional optical properties of an aerosol sample.
The accuracy of the Black Carbon Module has been validated through collocation testing alongside other best-in-class black carbon measurement instruments, including the Aerosol Magee Scientific AE33 and Teledyne 633. These tests have underscored the module's exceptional performance, affirming its role as a critical tool in the accurate detection and analysis of black carbon particles in the atmosphere.
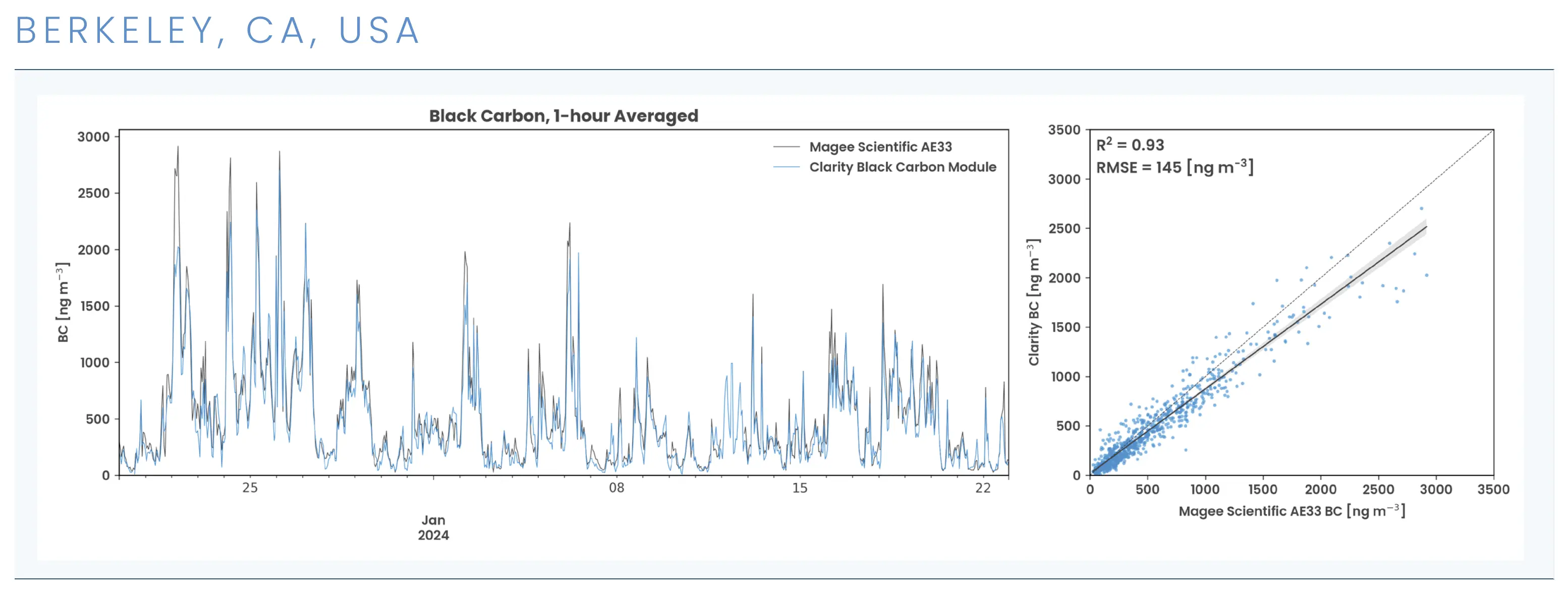

Similarly, source apportionment output from the AethLabs microAeth® instrument sitting at the core of the Clarity BC Module has been validated by users around the world. Data from a month-long collocation of a microAeth® MA350 and another best-in-class aethalometer (AE33) by scientists at the French National Institute for Industrial Environment and Risks (Ineris), Atmo Auvergne-Rhône-Alpes, and ADDAIR demonstrated a near 1:1 agreement in mean 15-minute biomass source-specific black carbon estimates (Mean slope = 1.01, r2 = 0.87).
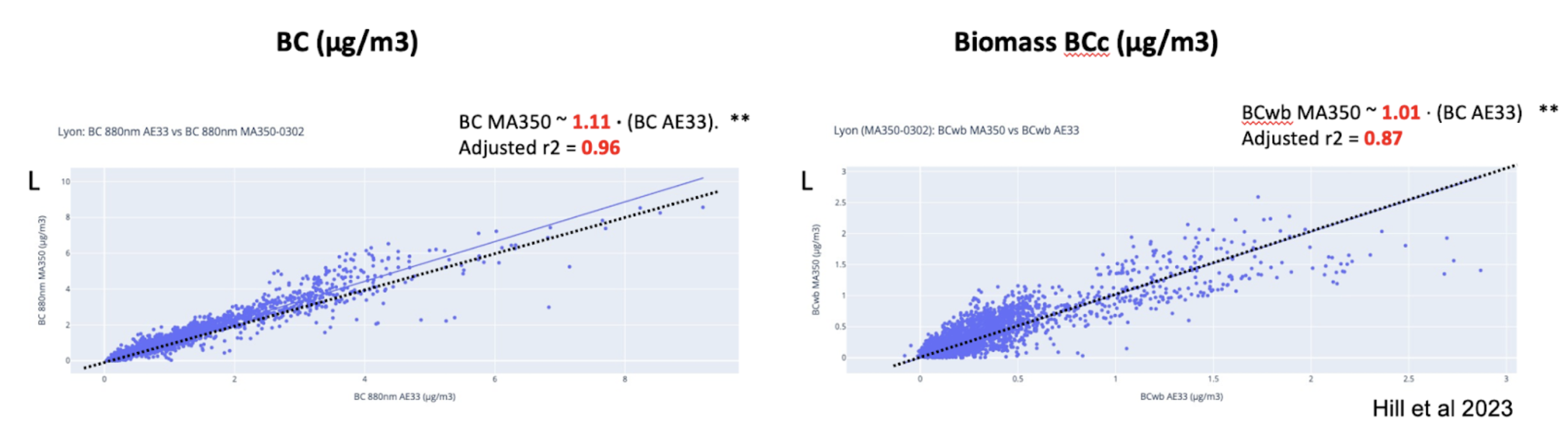
Beyond direct measurement tools, Chemical Transport Models (CTMs) can also be used to model black carbon concentrations. These models simulate the transport and transformation of pollutants in the atmosphere, providing estimates of the contribution of different sources, including black carbon. This method allows for a broader understanding of the distribution and movement of black carbon in the environment.
Satellite observations have also become a valuable resource in monitoring black carbon on a larger scale. Instruments on satellites, such as those on NASA's Terra and Aqua satellites, analyze the optical properties of aerosols in the atmosphere to estimate black carbon concentrations. This aerial perspective provides a global view of black carbon distribution, offering insights that complement ground-level measurements. In fact, AethLabs has been working with NASA JPL to implement another microAeth®-derived instrument to assist the Multi-Angle Imager for Aerosols (MAIA) satellite mission (launch date TBD) in its in-situ ground measurements of black carbon.
The variety of black carbon measurement techniques available allows scientists and policymakers to develop a more comprehensive understanding of black carbon's presence and impact on both local and global scales.
What are some compelling use cases for black carbon measurement?
As we’ve discussed, government and regulatory bodies tend to measure black carbon to understand its direct effects on health and climate and to track the progress of policy measures in mitigating black carbon emissions. While larger regulatory bodies focused on public health and environmental management often take the lead in measuring black carbon concentrations, their efforts are increasingly complemented by researchers, local & regional policymakers, and non-profit organizations dedicated to causes of environmental health, justice, and climate change mitigation.
One notable use case for black carbon measurement lies in its role as a strong real-time indicator of the adverse health effects associated with total PM2.5 exposure beyond the traditional measurement of total PM2.5 mass. As previously discussed, black carbon is increasingly observed to have more robust relationships with adverse health effects than PM2.5 concentration alone. For instance, the estimated increase in life expectancy associated with a hypothetical traffic abatement measure was found to be four to nine times higher when expressed in terms of black carbon particles (BCP) compared to an equivalent change in PM2.5 mass. This underlines the significance of black carbon as a useful tool for evaluating the health risks of air quality dominated by primary combustion particles.
Additionally, black carbon measurements can be used as a proxy for traffic combustion exhaust – a slurry of nasty stuff – helping to assess air pollution mitigation from traffic-related policies. These measurements are particularly crucial when examining vehicle emission factors, which are essential for preparing reliable emission inventories. These inventories, in turn, serve as strategic tools for effective air quality management.


As previously discussed in this blog post, wildfires in the North American West – a major contributor to local, regional, and global black carbon concentrations, and an important environmental justice issue – are increasing both in frequency and intensity, and are quick to erase gains in air quality made through diligent mitigation activities. This presents new challenges for air quality management and reduces our ability to predict the spatial and temporal distribution of black carbon emissions. A network of black carbon monitors can help fill the underlying knowledge gaps, inform more comprehensive black carbon emissions accounting and more effective black carbon control policies, and provide fire agencies with real-time feedback on fire & smoke behavior to protect firefighters and support efforts to manage wildland fire response.
Measurement of wildfire-related black carbon through multi-wavelength measurements can also be used to reduce exposure misclassification in epidemiological models that rely on black carbon as a proxy for other sources. A 2021 study in Denver, Colorado (USA) identified a 9% overestimation of traffic-related air pollution exposures along with reduced spatial gradients in exposure estimates during wildland fire smoke events with an overall effect of producing epidemiological bias toward the null (i.e. making true traffic-related air pollution effects look smaller than they really are). The authors of this particular study call out the potential benefit of multi-wavelength microAethalometer measurements in reducing such bias. An in-situ or mobile, fast-response/fast-deployment wildfire monitoring network could provide similar benefits for delineating residential biomass sources of black carbon from regional wildfire sources through increased spatial resolution.
Beyond their use in directly health-related endeavors, black carbon measurements facilitate the identification and mitigation of activities that significantly contribute to climate change. By understanding and quantifying black carbon emissions, organizations and policymakers can formulate targeted strategies to reduce the climate impact of combustion-related activities.
Taking action on black carbon air pollution starts with accurate air quality measurement
Scientists estimate that with the appropriate policy measures, readily available fuels and technologies could rapidly reduce black carbon emissions — it is already technically feasible to reduce global emissions by 70% by 2030 from 2016 levels. Armed with precise measurements, we can pave the way for informed decisions, targeted interventions, and a cleaner, healthier future for all.
If you’re interested in supporting this mission by taking black carbon measurements, you can now order a Black Carbon Module from Clarity for solar-powered, cellular-connected measurements built on AethLabs’ best-in-class black carbon measurement technology.